What is a supernova? Are all stars destined to go through it?
One of the most impressive fireworks displays of the universe.

A supernova is one of the most spectacular fireworks displays in the universe. The last gasp of a sufficiently large star in its death throes, the star’s explosive finale often becomes the brightest point of light in an entire galaxy in less than a second and can remain so for several weeks afterward before fading away into a spectacular nebula spread across dozens if not hundreds of light-years.
What causes such a violent end to the life of a star, though, and is a supernova the fate of every star? Can a supernova produce a black hole?
And what about our own Sun? Is it, too, destined for a spectacular final bow several billion years from now? Fortunately, supernovas are some of the most actively studied celestial events, so there is a lot that we know about them, even as they keep some secrets to themselves, for now.
What is a supernova?
A supernova is a key stage in the “dying” process of certain stars. There are two broad classes of supernova, each the product of a different process and conditions.
The Type II supernova is the kind that most people think about when they think about supernova; the classic explosion of a dying star brought on by something known as core collapse. There are several stages that most stars pass through over the course of their lives, and as far as supernovas are concerned, we’re talking principally about main sequence stars like our Sun, only larger.
Stars below the level of the main sequence, like red dwarfs, are the proverbial tortoise to the main sequence star’s hare and can steadily burn their limited hydrogen fuel judiciously for trillions of years while larger stars like our Sun burn themselves out after several billion years or less.
It is at this late stage of a main sequence star’s life that a supernova becomes possible. While not every star in the main sequence will go supernova, those that do will first pass through a “giant” phase after they exhaust their hydrogen reserves and instead start fusing helium and residual heavier elements like oxygen, silicon, and carbon rather than hydrogen (limited fusing of these heavier elements takes place in young stars as well, but doesn’t account for their primary fusion reactions).
These nuclear reactions will sustain a star for another few billion years after it transitions to its giant phase, but eventually, the fusion process climbs up the periodic table to the point that it starts fusing lesser elements into iron or even heavier elements like gold. Once you start fusing atoms into iron, the process actually absorbs energy instead of producing it, so once a star starts to grow a heavy iron core, its end approaches at an accelerating pace as heavy metal fusion sucks up whatever energy is being generated elsewhere.
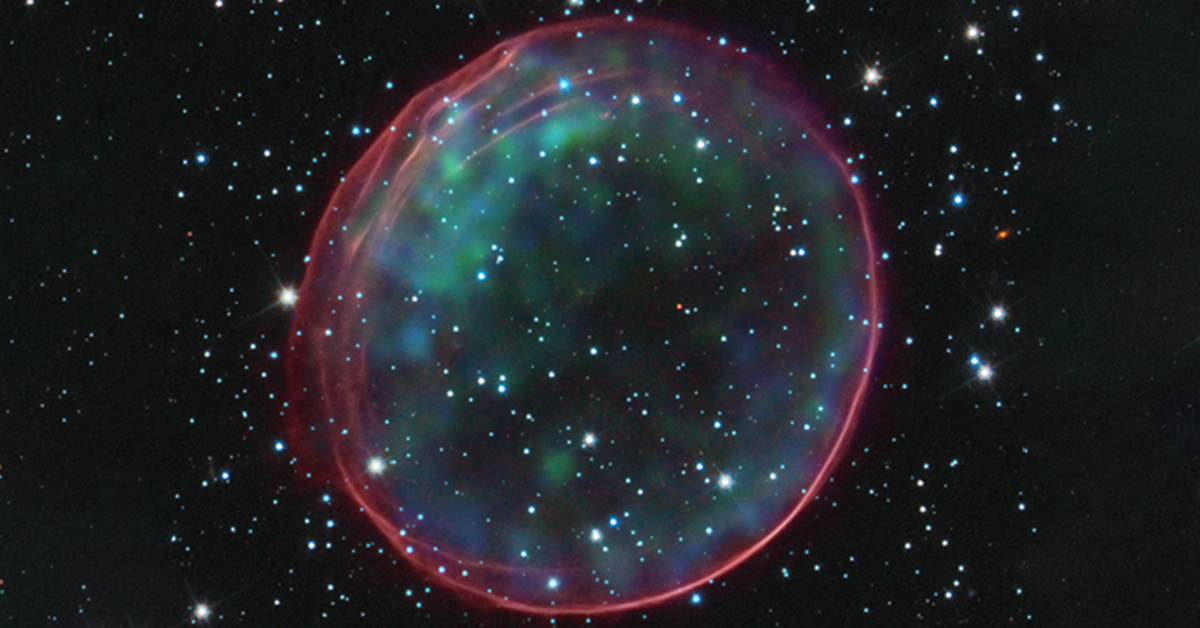
This becomes a huge problem for the star because it’s not like it’s lost any mass over the preceding billions of years. Its heavy outer layers of gas and plasma, previously held aloft by the energy produced by fusion reactions beneath, suddenly cross a tipping point and their mass is too great for the star to hold up. These layers rapidly collapse in toward the star’s core, kicking off the supernova process.
The other class of supernova, a Type I supernova, is less well-understood and is actually subdivided further into Type Ia, Ib, and Ic supernovas. Astronomers are fairly comfortable with the mechanics of the Type Ia supernova, which is thought to occur in binary star systems with at least one white dwarf star, so we’ll use that as a representative example.
As a white dwarf co-orbits its companion star, it steadily sucks material off of it into an accretion disk around itself. There is an upper bound of mass, known as the Chandrasekhar limit, under which a white dwarf can accrete material without becoming unstable (about 1.44 solar masses). In the case of a Type Ia supernova, a white dwarf accretes material from its companion that pushes it over this mass limit and it can no longer support its own mass.
Unlike a nova—where a white dwarf accretes a more modest amount of material below this limit and produces a relatively mild explosion of energy from the additional mass but otherwise remains intact—in a Type Ia supernova, the white dwarf destabilizes in an explosive fashion and destroys itself in the process.
Type Ib and Ic supernovas are very similar to Type II supernovas in that they are a function of core collapse after the stars have exhausted their fuel to sustain fusion reactions in their core. The only real difference, in this case, is fairly academic; Type Ib and Type Ic supernovas occur during a core-collapse event in stars that have shed their outermost layer of hydrogen, or their outermost hydrogen layer, and a substantial part of the helium layer below that, for Type Ib and Type Ic, respectively.
Which stars go supernova?
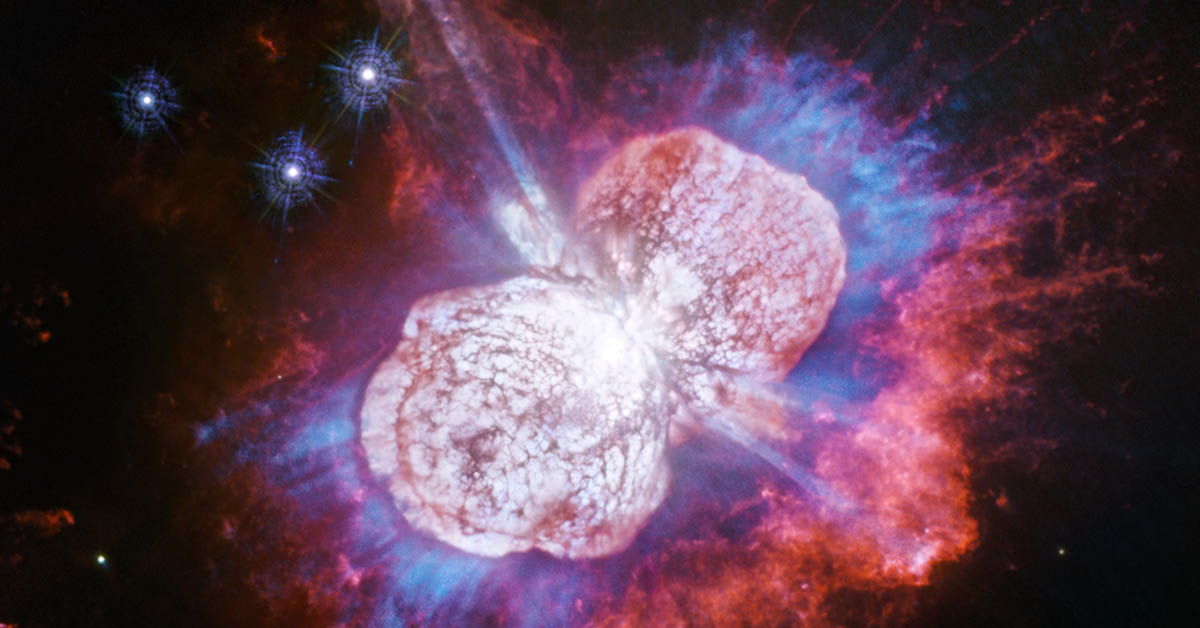
The mass of a star plays a huge role in whether a star will go supernova at the end of its lifecycle.
Generally speaking, stars heavier than eight solar masses are candidates for supernovas, though even at these enormous masses, particular circumstances can sway things in one way or the other.
In 2008, a star with 25 solar masses exhausted its fuel and should have progressed to its final supernova event, but instead shuffled off the galactic stage with barely a recordable blip of activity, most likely collapsing directly into a black hole.
Computer simulations had previously shown researchers that the stars they thought should have blown up didn’t, and still there isn’t a clear explanation why some stars in the eight to 30 solar mass range explode in supernova and others seem to fizzle out.
Further investigation points to a range of eight to 17 solar masses as the most likely to collapse into a supernova, with stars between 17 and 30 solar masses often taking a less flashy end-of-life path, but not always.
Can a supernova create a black hole?
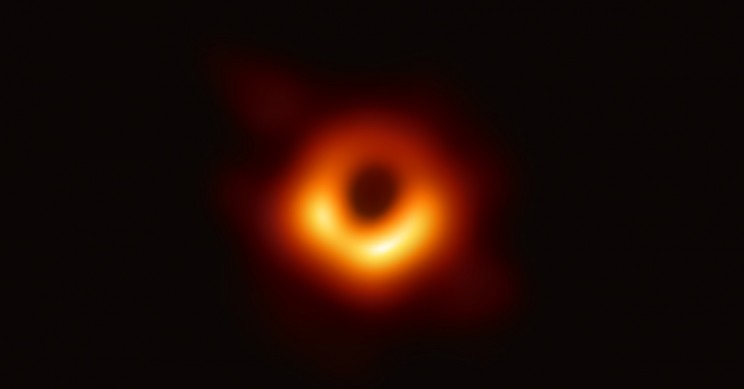
On the other end of the eight to 30 solar mass range, we have those stars heavier than about 17 solar masses. Stars that are between 30 and 100 solar masses are simply too big to be able to sustain their own mass during a core-collapse event and so will continue to collapse inward toward infinite density, creating a singularity.
The so-called Schwarzschild radius determines the location of the new event horizon around this singularity, and a black hole is subsequently produced. What happens between 17 and 30 solar masses is more tricky, however, and there is a lot of academic debate about how stars this size go supernova (or why some don’t produce a supernova at all). There is solid evidence that at least some of the stars in this range collapse directly into stellar black holes and skip the supernova part altogether.
What’s more, a black hole doesn’t necessarily need to be produced immediately upon stellar collapse. You may sometimes have supernova eruptions that eject a large amount of stellar material out into space, but not with enough force to push it beyond the gravitation influence of the remaining stellar core (usually a neutron star).
In these cases, the material from the outer layers begins to accrete back onto the neutron star, and after accreting a critical mass of supernova remnant material, the extra mass can push borderline neutron stars that barely missed becoming black holes during the prior core-collapse phase over the line into becoming black holes.
What happens during a supernova?
So what actually happens inside a star in the second or two it takes for the star to explode in a supernova? The actual mechanics are still debated, but in the case of a Type II supernova, we know that the core’s lack of energy-producing fusion means that the core itself can no longer hold itself up, much less the stellar material in the outer layers.
When this happens, the core undergoes an even more rapid collapse than that of the incoming outer layers. If the core is under three solar masses, then the collapse produces such immense pressure that the inward rush of core material overcomes the quantum forces that keep electrons in orbit around an atomic nucleus and simply smashes electrons into the protons, fusing them both into a neutron.
This happens all throughout the core of the star until all that’s left is a fantastically dense ball of neutrons so tightly packed together that the resulting rapidly-spinning sphere might be only about 12 miles across with a mass equivalent to several Suns. For context, a spoonful of this star would weigh about 50 billion tons on Earth.
The supernova is only half done, however. We still have all that infalling stellar material rocketing in towards the core at speeds of hundreds of kilometers a second. All of this infalling material is converging on the second densest material in the universe, so the majority of this massive star’s mass will end up violently bouncing off it as surely as a racquetball rebounds off the concrete wall in a schoolyard.
This rebounding off the inner neutron star is what gives a supernova its explosive umph. The energy released in this process, which can take just a few seconds from start to finish, is greater than all of the energy the Sun will have produced over a 10 billion years period. Not all of the star’s outer material will hit the core and bounce off it, though; it is a rather tiny target, after all. A lot of it will miss the core entirely and get slingshot back out into space by the incredible gravitational energy generated by its collapse towards the core.
Between the rebound and slingshot effects on the outer layer, an enormous shockwave erupts from the center of the now-dead star that blows the outer layers out into space at incredible speed. This, combined with the release of radiation from the material rebounding off the neutron star, is the actual Type II supernova explosion that we see.
Still, even with all of that explosive force, computer models stubbornly refuse to recreate these explosions consistently, leading many to look for what other processes might be contributing to the supernova. One theory that is gaining increasing acceptance is a neutrino-driven model for a supernova. All stars produce neutrinos naturally, but during the core-collapse event, the violence at the core produces a lot of neutrinos all at once and shoots them off in all directions.
Normally, neutrinos are nearly massless particles that very rarely interact with ordinary matter, but the circumstances of core collapse are so extreme that the neutrinos may be produced in such great quantity that they actually have the effect of sustaining the explosive shockwave that pushes the outer layers of a collapsing star into space, enabling the spreading supernova remnant to escape the gravity of the neutron star.
This hasn’t been conclusively proven, but the chemical compositions of some observed supernova remnants line up very neatly with the neutrino-driven model. Concentrated bubbles of titanium and chromium in iron-rich supernova remnants should be created by large pockets of trapped neutrinos producing plumes of high-entropy material.
Researchers have identified exactly these kinds of titanium and chromium “bubbles” in some supernova remnants, providing compelling evidence that neutrinos play some role in sustaining the shockwave required for at least some Type II supernova explosions.
Speaking of explosions, this process doesn’t leave the core unscathed either. The pressure being put on the neutron star in the center of the explosion isn’t consistently distributed. Given the pressures involved, this disequilibrium can be enormous in absolute terms, meaning a neutron core can actually be shot out of the supernova as if it was fired from a gun, hitting speeds of millions of miles an hour—a velocity fast enough that regardless of its trajectory is likely to shoot the stellar core clear out of the galaxy.
Still, for as much as we know about core-collapse supernovas, there’s still plenty of room to be surprised. Catching an actual supernova from start to finish is a very hard thing to do, and it was only in 2020 that astronomers were able to watch the entire core-collapse supernova process play out in real-time. It turned out to be even more violent than the researchers were anticipating.
“It’s like watching a ticking time bomb,” said Raffaella Margutti, an associate professor of astronomy at UC Berkeley who was the senior author of the 2022 paper describing the event. “We’ve never confirmed such violent activity in a dying red supergiant star where we see it produce such a luminous emission, then collapse and combust, until now.”
The red supergiant whose death resulted in the SN 2020tlf supernova was about 10 solar masses and erupted unexpectedly with bright emissions and violent explosions of gas along its surface immediately preceding its collapse. The new observation deepens the mystery of the kinds of structural changes taking place below the dying star’s surface.
“I am most excited by all of the new ‘unknowns’ that have been unlocked by this discovery,” Wynn Jacobson-Galán, an NSF Graduate Research Fellow at UC Berkeley who was the lead author of the study, said. “Detecting more events like SN 2020tlf will dramatically impact how we define the final months of stellar evolution, uniting observers and theorists in the quest to solve the mystery of how massive stars spend the final moments of their lives.”
But what about a Type I supernova? We aren’t really sure what actually causes the massive explosion in these types of supernovas, but it’s suspected that once a white dwarf crosses that critical 1.44-solar-mass threshold, nuclear fusion actually reignites in the core and the white dwarf starts fusing carbon and oxygen into heavier elements.
The energy produced by this might be triggering a runaway nuclear fusion reaction that effectively turns the entire mass of the white dwarf into a thermonuclear bomb, which then blows itself apart in a supernova. Likewise, the merger of two white dwarfs whose combined mass exceeds the 1.44-solar-mass limit might also produce a supernova for the same reason.
Will our Sun go supernova when it dies?
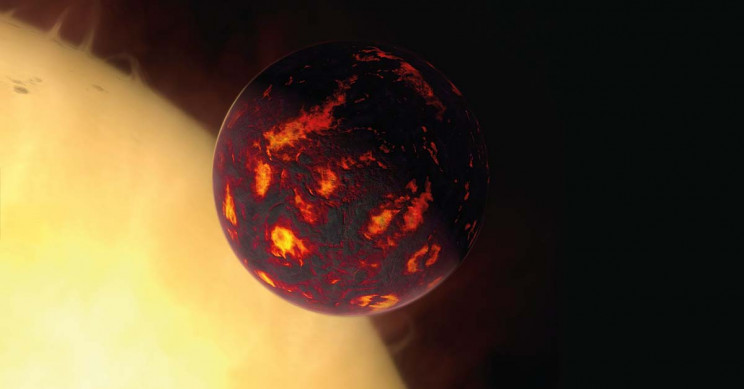
Anyone hoping to see our Sun similarly explode at the end of its life, you’re going to be disappointed. Our Sun falls below the eight-solar-mass lower bound for producing a supernova, and so it is ultimately destined for a much more casual exit when it exhausts its fuel.
Main Sequence stars below eight solar masses will eventually swell into red giants (likely consuming any inner planets in the process), but that will likely be the most violent part of the process. When these red giants exhaust their helium fuel, they don’t explode violently, but instead, they quietly shed their outer layers out into space and leave behind their white-hot inner core, a white dwarf.
The outer layers then spread out into what we call a planetary nebula with the white dwarf in its center. This process has produced some of the most famous nebulae in the universe, like the Crab Nebula. That, ultimately, is the fate of the Sun when it exhausts its fuel a few billion years from now. It might not be an explosive end, but the result should still be spectacular in its own way.
What are some of the most famous supernovas?
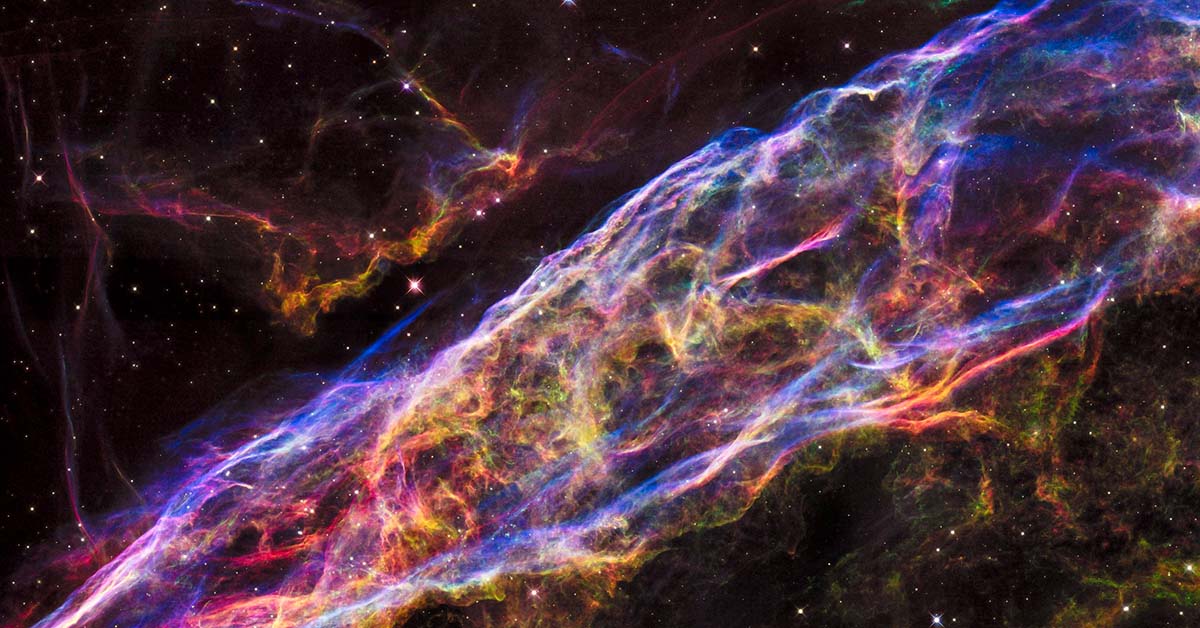
Fortunately, because supernovas are so bright and energetic, we’ve actually observed a lot of supernovas in the past, and astronomers through the centuries even kept pretty good records of their observations.
One of the most famous was the “Guest Star” observed by Chinese and Japanese astronomers in 1181 CE. It was about as bright as Saturn and remained visible in the night sky for several months before eventually fading away. Of all the supernovas recorded in the past 1,000 years, only the Chinese Guest Star has yet to be identified by astronomers, a mystery stretching back some 900 years.
We now know that this was actually a Type Iax supernova (a sub-type of Type 1a) involving Parker’s Star, thanks to the detailed astronomical records kept by the Chinese astronomers. By looking at the recorded position of the Guest Star in the night sky, astronomers were able to home in on the nebula Pa30, which is believed to be the result of a supernova produced by the merging of two white dwarf stars in a binary system. The nebula is expanding rapidly, but researchers were able to calculate this velocity and work backward to the source of the nebula and the rough date of the supernova that produced it.
“The historical reports place the guest star between two Chinese constellations, Chuanshe and Huagai,” said Albert Zijlstra, a professor of astrophysics at the University of Manchester. “Parker’s Star fits the position well. That means both the age and location fit with the events of 1181.”
Another very important supernova is known as Tycho’s supernova. In 1572, the Danish astronomer Tycho Brahe observed the appearance of a bright new object in the constellation Cassiopeia. Unlike other objects like comets that can suddenly appear before disappearing weeks later, Tycho was able to demonstrate that this “new star” was far beyond the Moon and as distant as many of the other stars in the night sky.
This showed that rather than a static firmament of stars set in place at the beginning of time by God, the stars were actually subject to change. While this definitely upset plenty of traditionalists, Tycho’s supernova was an important milestone in the development of the nascent Scientific Revolution in Europe.
Recently, the phenomenon known as a light echo—where light bounces off dust and other space material and gets reflected back in a similar way to sound waves bouncing off hard walls—was observed in the remnant of Tycho’s supernova. Astronomers were able to determine that this reflected light was actually the same light produced by the very supernova that Tycho observed in 1572.
“I think it is cool that I can look in the sky and still see the same light that Tycho did, at the time of his truly revolutionary discovery,” said Texas A&M astronomer Nicholas Suntzeff. “Tycho was the astronomer who proved Aristotle wrong. Aristotle believed—and it was taught in all Catholic and Protestant schools for 1,500 years—that the Earth was at the center of the Universe, and all things variable were between the Earth and Moon. This supernova proved that theory wrong and quickly led to a freedom of thought in science, which is central to the way science operates today.”
So, in a way, a supernova helped kick off the early Scientific Revolution during the Middle Ages in Europe, which seems appropriate. So much of what we see in the universe, from enormous nebulas rich in heavy elements to the very physical structure of the Milky Way itself owes itself to the incredible violence of supernovas through the eons, so why wouldn’t they make themselves felt culturally too?
As Carl Sagan rightly pointed out, all of the heavy elements in our bodies, like carbon, calcium, and iron, were forged in the furnace of some red giant star that exploded somewhere in the universe billions of years ago. Our entire solar system likely wouldn’t exist without a helpful push from a nearby supernova, and any element bigger than helium can likely trace itself back to a supernova at some point.
A supernova, then, is a kind of thread that stitches one age of the cosmos to the next, a vital process in the life of the universe that is both spectacular and awe-inspiring. It’s little wonder then that we will never cease to be fascinated by them.